Established and Emerging Therapies in Acute Spinal Cord Injury
Article information
Abstract
Acute spinal cord injury (SCI) is devastating for patients and their caretakers and has an annual incidence of 20–50 per million people. Following initial assessment with appropriate physical examination and imaging, patients who are deemed surgical candidates should undergo decompression with stabilization. Earlier intervention can improve neurological recovery in the post-operative period while allowing earlier mobilization. Optimized medical management is paramount to improve outcomes. Emerging strategies for managing SCI in the acute period stem from an evolving understanding of the pathophysiology of the injury. General areas of focus include ischemia prevention, reduction of secondary injury due to inflammation, modulation of the cytotoxic and immune response, and promotion of cellular regeneration. In this article, we review established, emerging, and novel experimental therapies. Continued translational research on these methods will improve the feasibility of bench-to-bedside innovations in treating patients with acute SCI.
INTRODUCTION
Spinal cord injuries (SCIs) are devastating traumatic events in the lives of patients, often resulting in severe and/or permanent neurologic disabilities. Nearly half a million people are living permanently disabled in the United States due to traumatic SCI, and 12,000–15,000 patients per year incur new injuries [1,2]. Further, the incidence of SCI varies by country with most developed countries reporting incidences of 20–50 per million [3,4]. The event can result in an assortment of immediate sequelae and long-term complications including loss of motor and sensory function, loss of autonomic function, and increased risk of medical complications and death [5].
As traumatic SCI more commonly occurs in young, healthy adults, the associated quality-adjusted life year and economic loss can be immense [6]. Despite recent gains in understanding of the pathogenesis and improved acute management of SCI, there remains an ongoing need to investigate novel molecular targets and therapeutics for treatment in the acute injury period, with the goal of maximizing patient recovery and preventing chronic manifestations of the injury. While the timing of surgery for decompression and stabilization of acute SCI has been well studied [7], the evidence for most medical treatments of the acute neurological injury are less well supported. This review discusses the established current management strategies and expands on emerging therapeutic strategies for acute SCI. Additionally, we discuss an updated review of experimental therapies, from bench to bedside.
MECHANISM OF INJURY IN SPINAL CORD INJURY
SCI can result either from high-energy trauma (e.g., motor vehicle accidents, diving, sports) or lower impact trauma, which often occurs in the presence of underlying degenerative or congenital spinal spondylosis. The cervical spinal cord is the most commonly affected region due to the lack of bony and muscular protection relative to thoracic and lumbar segments [8]. The mechanism of spinal cord damage is generally divided into primary and secondary processes. Primary injury encompasses the initial insult, such as compression, penetrating injury, or strain on neural tissue or vascular structures. Subsequent perfusion disruption progresses to local hypoxia and can be worsened by systemic vasogenic shock. Hypoxic cell death induces the multifaceted, progressive process of secondary injury during the following hours to weeks. Ischemia caused by primary injury activates release of vasoactive proteins and cytokines, and the resulting edema and inflammation promote cytoskeletal and mitochondrial damage and immune cell infiltration. The zone of injury then expands due to reactive oxygen species (ROS) accumulation and oxidative damage, lactic acidosis, fibrin and platelet accumulation, toxic excitatory neurotransmitter release, and axonal demyelination, leading to progressive tissue death and scarring.
CLINICAL PRESENTATION
Initial management of patients with suspected acute traumatic SCI follows established trauma guidelines to manage the airway, breathing, and circulation. Immobilization is necessary to prevent further possible damage in all patients with suspected SCI (characterized, for example, by polytrauma, neck/back spinal pain, dysesthesia, loss of consciousness, or a mechanism of injury with the potential to cause an SCI) [9-11].
Patients with SCI can present with either complete injury, defined as a lack of motor or sensory function in the anal and perineal region (representing the lowest sacral segments S4–S5), or incomplete injury with varying degrees of motor and sensory function caudal to the level of the injury [12]. The American Spinal Injury Association (ASIA) scoring system is used to stratify injury severity, ranging from complete injury (ASIA-A) to normal neurological exam (ASIA-E), to standardize assessment and treatment of SCI [2]. In addition, patients can present with spinal shock, an often-transient physiologic depression of spinal cord function secondary to cell damage which can be tracked with progressive recovery of anatomical reflexes including the bulbocavernosus, anal cutaneous, and plantar reflexes [12]. Spinal shock and medication effects may obscure accurate ASIA scoring, particularly in the first 48–72 hours after injury [13]. This process is distinct from neurogenic shock, a condition of autonomic dysregulation from injury to spinal levels C1–T6 and/or associated sympathetic ganglia, which is characterized by persistent hypotension, bradycardia, and hypothermia [12].
ESTABLISHED ACUTE MANAGEMENT STRATEGIES
Computed tomography of the entire spine is indicated in the initial evaluation of traumatic SCI patients [14]. ASIA motor scores and neurologic level of injury have the most consistent prognostic value regarding outcome [15]. Magnetic resonance imaging has utility for guiding surgical intervention and prognostication and should be performed when available but should not delay surgical treatment in a neurologically declining patient [12,15]. Standardization of magnetic resonance changes of increasing severity in acute SCI is increasing, with the BASIC score serving as one example [16]/ Surgical candidacy should be promptly determined through assessment of a worsening neurological examination along with signs and symptoms of instability and progressive compression.
Direct spinal cord compression is the most frequent mechanism of SCI and can progress following the initial injury, prompting a need for emergent surgical decompression. A meta-analysis including 16 studies involving nearly 4,000 patients found that patients who receive early spinal surgery (< 24 hours following injury) experience greater neurological recovery, shorter length of stay, lower hospitalization costs, and a lower incidence of complications than those who undergo surgery > 24 hours following injury [17]. Another recent multicenter clinical trial compared early and delayed surgical treatment (defined as ≤24 hours after injury and after ≥ 2 weeks of conservative therapy, respectively) and assessed motor recovery using the ASIA motor score, spinal cord independence measure, and independent ambulation capacity for 1 year. The study found that the 2 treatment paradigms were not significantly different; however, motor recovery at 2-week, 3-month, and 6-month follow-ups were improved with early surgical treatment, indicating accelerated recovery [18]. These results align with several large, well-documented trials and underscore the importance of early decompression to improve potential for motor recovery following SCI [19-21].
Medical management has an essential role in the optimization of patients with SCI [12,22]. Hemodynamic control is crucial in the early stages of acute SCI to reduce potential ischemic injury, with goal mean arterial pressures (MAPs) of 85–90 mmHg for 5–7 days suggested for improved functional outcomes [23]. Other crucial tenets of management include prevention of pulmonary (i.e., pneumonia), gastrointestinal (i.e., bowel obstruction), and urologic (i.e., dysautonomic uropathy) complications caused by loss of voluntary and autonomic control [12,14]. As such, current multidisciplinary clinical studies are examining, for example, the use of low versus high tidal volume mechanical ventilation in patients requiring intubation in acute SCI (NCT04912583) and the use of early epidural and sacral nerve stimulation as an adjunct for improving bladder function (NCT03083366) [24,25]. The utility of methylprednisolone (MPSS) administration has been discussed extensively in the literature and, while initially thought to improve outcomes based on the early North American Spinal Cord Injury Study (NASCIS) results, subsequent large studies have not demonstrated this benefit [26-28]. Moreover, a consistently increased risk of steroid-associated complications including infection was found. Recent animal studies have found that high-dose steroid administration may actually increase the extravasation of plasma components after SCI and can enhance tissue swelling and edema [29]. Therefore, routine administration of high-dose steroids is no longer recommended; a short course may, however, be considered in young, otherwise healthy adults presenting <8 hours from injury based on expert consensus [27,28,30]. Early physical rehabilitation, nutritional optimization, and mental health consultation are indicated, and have been shown to improve functionality and quality of life [31-33]. These therapies continue to be studied, for example, in clinical trials assessing the effects of interval versus continuous aerobic training on autonomic dysreflexia in the acute phase of SCI (NCT05061160).
EXPERIMENTAL ACUTE MANAGEMENT STRATEGIES
Emerging therapeutic strategies for acute SCI can be classified by the biological mechanism which they are designed to address, prevent, or reverse. Mechanisms largely discussed in the literature to date can be grouped into categories as described below based on the current understanding of SCI pathophysiology (Fig. 1, created with BioRender.com).
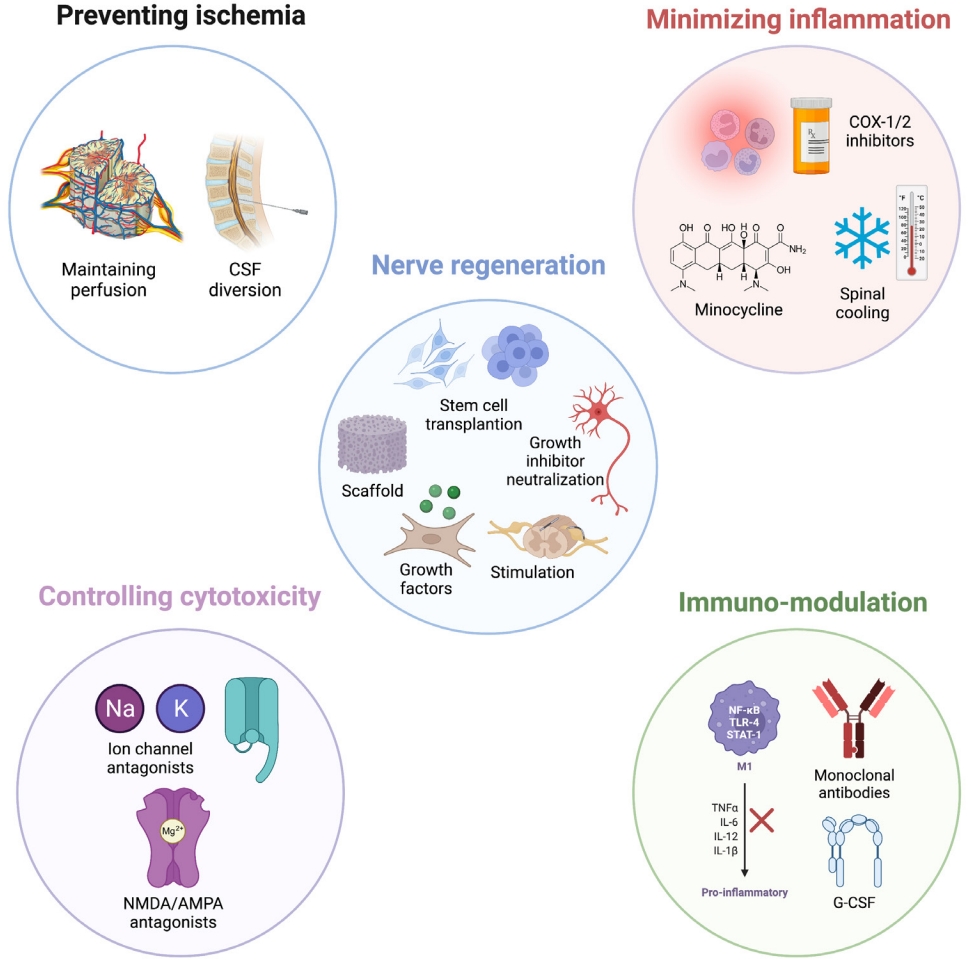
Emerging therapies for acute spinal cord injury. CSF, cerebrospinal fluid; COX, cyclooxygenase; NMDA, N-methyl-D-aspartate; AMPA, α-amino-3-hydroxy-5-methyl-4-isoxazolepropionic acid; TNF, tumor-necrosis factor; IL, interleukin; G-CSF, granulocyte-colony stimulating factor.
1. Preventing Ischemia
In the early stages of SCI, bleeding caused by disruption of local vasculature can lead to ischemic hypoxia within spinal grey matter. Hypoxia in these highly metabolically active structures leads to neuronal dysfunction, demyelination, and the initiation of apoptosis and necrosis [34]. Uncorrected ischemia can cause secondary damage due to the cumulative effects of cellular swelling and the initial macrophage-mediated inflammatory response which may last between 3–24 hours following the inciting event [34]. Furthermore, without proper blood flow, the highly vascular and regenerative astrocytic environment is less equipped to repair axonal and neuronal damage. Maintaining adequate perfusion systemically and within the remaining spinal vascular beds is essential for mitigating the pathological consequences of SCI.
States of neurogenic shock can further compound the ischemic burden. Prompt correction of hypotension with fluid resuscitation, transfusion, or traditional vasopressors can be difficult due to the disruption of local capillary auto-regulatory mechanisms. Agents including dopamine and midodrine have been attempted in clinical trials with varying levels of success and no high-level evidence supports the use of one pressor over another [35]. When compared with norepinephrine, dopamine maintained MAP to a similar degree but was associated with a higher intrathecal pressure (ITP) and consequentially decreased spinal cord perfusion pressure (SCPP); dopamine use was also associated with higher rates of vasopressor-associated complications [22]. A handful of case reports and small clinical trials of midodrine note successful acute maintenance of blood pressure and orthostatic symptoms with no serious adverse effects but lacked long-term follow-up [36]. Importantly, hypertension should be avoided in acute SCI to lower the risk of hyperemia and hemorrhage.
Cerebrospinal fluid (CSF) diversion has potential to alleviate ischemia caused by venous edematous leakage following SCI through the reduction of ITP. This strategy was extrapolated from the cranial trauma practice of ventriculostomy placement and the vascular surgery literature’s investigation of the use of lumbar drains in aortic dissection to support cord perfusion [37]. A preclinical study utilizing a pig SCI model previously demonstrated a significant and sustainable improvement in blood flow and SCPP with CSF drainage [38]. An early human study was undertaken with 22 patients randomized to undergo CSF drainage within 48 hours of injury versus not [39]. Lumbar drainage was not associated with significant side effects but also did not demonstrate definite neurologic benefit, though the authors cited a low power as one potential cause. Subsequent larger studies demonstrated more significant benefits with adequate safety profiles; however, a well-defined effect remains to be reproducibly demonstrated [40]. Patch duraplasty is another method of lowering ITP on the injured cord and can be performed during initial surgical decompression [41]. One study found that laminectomy with patch duraplasty resulted in lower ITP and improved spinal perfusion pressure compared with laminectomy alone in acute SCI; additionally, ASIA impairment and bowel/bladder function was improved in the duraplasty group. However, low power contributed to a lack of statistically significant differences in clinical outcomes [42]. These studies underscore the overall difficulty in performing well powered and large-scale human trials on acute SCI.
Hyperbaric oxygen (HBO), when administered early, may counteract spinal cord ischemia and hypoxia, potentially reducing apoptosis, oxidative damage, inflammation, and edema, and promoting angiogenesis and autophagy. Recent clinical studies have initiated therapy within 9–20 hours of injury with varying time-courses, demonstrating varying degrees of neurologic improvement [43]. Technical parameters (onset, duration, frequency, and pressure) and evaluation of benefits in more heterogenous samples of SCI presentations remain to be investigated. Two clinical studies are ongoing in China (NCT03112941) and Austria (NCT03101982).
2. Minimizing Inflammation-Induced Secondary Damage
The inflammatory cascade occurring hours to days following the primary of insult of SCI likely contributes to worsened neurological outcomes [34]. This secondary damage acts on the injured spinal cord through cellular and systemic factors that worsen compression, ischemia, and scarring [44]. Synthesis of proinflammatory prostaglandins and cytokines, calcium-dependent nitric oxide, opioid peptides, and necroptotic factors have been shown to augment inflammatory secondary damage in the acute stages of injury. To address this component of the injury, several commonplace pharmacological agents have demonstrated efficacy in maintaining blood flow and protecting from cellular damage in preclinical studies [34]. Cyclooxygenase inhibitors including ibuprofen and meclofenamate as well as nonselective opioid antagonists such as naloxone have been shown to improve blood flow and enhance functional results in animal spinal cord contusion models [45-48]. Still, few trials have attempted to establish the effects of these agents in human SCI. In 1990, NASCIS II found no neurologic benefit with the administration of naloxone throughout the first 24 hours after SCI compared to placebo, although those recommendations are being revisited more broadly [26]. A phase-I clinical trial involving 12 patients investigating the use of ibuprofen administration in acute SCI is in progress (NCT02096913).
Minocycline, a tetracycline antibiotic, has demonstrated multiple neuroprotective effects against secondary injury progression in SCI [34]. Minocycline impedes generation of proinflammatory cytokines (IL-1beta, tumor-necrosis factor [TNF]-alpha, cyclooxygenase-2), hinders expression of proapoptotic caspase-1/-3, and inhibits inducible nitric oxide synthase [49,50]. Successful preclinical studies showed enhanced tissue sparing and motor recovery within 3–4 weeks following SCI in mice [51,52]. A phase-II clinical trial demonstrated that patients with acute incomplete cervical SCI can benefit from early minocycline administration [53]. The study reported a 14‐point ASIA motor score recovery in patients receiving minocycline administration for 7 days following injury compared with placebo [53].
Spinal cooling is a nonpharmacologic method that has been explored for its potential to ameliorate biochemical secondary injury. One study utilized a combination of surgical decompression, steroid administration, and regional hypothermia in 20 patients and saw an improvement from initial ASIA-A impairment in 13 patients [54]. The lack of randomized comparative control arms in this study and the few other case reports/small clinical series available limit the generalizability of spinal cord cooling in acute SCI; however, the promising initial results and absence of reported adverse effects may warrant further study [12].
3. Controlling Cytotoxic Response
The acute cytotoxic response to SCI is another targetable pathomechanism. Calcium, sodium, and potassium ion dysregulation as well as release of high levels of glutamate from damaged neural tissue can cause excitotoxicity and oxidative damage. Signaling cascades that lead to ROS formation and lipid peroxidation increase the likelihood of neuronal cell death. Inhibiting free radical formation through various antioxidants including cyclosporin A, vitamins C, D, and E, selenium, lithium, polyethylene glycol-superoxide dismutase, and OXR1 gene-enhancing therapy, have all shown promise in maintaining cell viability in animal models [34]. One 16-participant, phase-I/II clinical trial of lithium in China (NCT01471613) is the first human trial to incorporate one of these agents as an adjunct in acute management strategies.
Uncontrolled activation of voltage-gated sodium channels has been hypothesized as an important step in the cytotoxic response leading to secondary spinal trauma. Riluzole is a benzothiazole that inhibits voltage-gated sodium channel-mediated glutamate release and recently gained approval for the treatment of amyotrophic lateral sclerosis. Previous animal studies have documented reduced neuronal loss and sensorimotor improvement with Riluzole administration following SCI, likely through inhibition of glutamate release and subsequent reduction of calcium-signaling induced apoptosis reduction [55]. A successful phase-I trial involving 36 patients found that 50 mg of Riluzole orally every 12 hours for 14 days enhanced motor recovery in cervical SCI at 3 months postinjury compared with a matched control group [55]. Similar motor enhancement was not found at 6-month follow-up. A transient increase in liver enzymes in the Riluzole group prompted questions of pharmacotoxicity, however its safety profile has yet to be presented in larger studies. The AO Spine North America Research Network is currently conducting the Riluzole in Acute Spinal Cord Injury Study, a multicenter randomized trial designed to evaluate the therapeutic application of Riluzole in acute SCI.
Antagonism of α-amino-3-hydroxy-5-methyl-4-isoxazolepropionic acid/ N-methyl-D-aspartate (NMDA) receptors has been postulated as a mechanism of reducing excitatory amino acid (glutamate) toxicity in the hyperacute phase of SCI. These neurotransmitters reach toxic levels within 15 minutes of injury and typically peak for less than 120 minutes [34]. Gacyclidine, an NMDA receptor antagonist, has been evaluated in a phase‐II double‐blind, randomized evaluation of 280 patients with SCI [56]. However, this study revealed no significant improvement in ASIA scores compared with placebo treatment. Still, other NMDA antagonists such as magnesium have been shown in preclinical studies to reduce excitotoxicity and inflammation. Organized phase-I/II clinical trials have yet to be completed examining this therapy in acute SCI.
Calcium-channel blockers (CCB) can significantly reduce the pathological influx of calcium following SCI and improve perfusion through regulation of vascular smooth muscle tone. The utility of nimodipine has been studied, most notably in a French clinical trial of 106 patients with SCI randomized to receive MPSS, nimodipine, MPSS and nimodipine, or placebo within 8 hours of injury for 7 days duration. Neither additional neurologic benefit nor significant adverse effects in the nimodipine treatment group versus control were noted [27]. Still, concerns for systemic hypotension in the context of injured auto-regulatory mechanisms in cervicothoracic SCI should prompt caution when considering CCBs during acute management. Finally, potassium channel antagonists such as the fast potassium channel blocker fampridine may have potential therapeutic benefit through improvement of axonal conduction in SCI and are currently being tested in phase-III trials [34].
4. Modulating the Immune Response
Immunomodulative therapies are currently under investigation for the treatment of acute SCI. Peripheral immune cells including macrophages, neutrophils, and T cells trigger an inflammatory response following injury that can last several days. Importantly, this response can cause cavitary lesion growth, further damaging surrounding spinal cord. Inflammatory cytokines including IL-1beta, TNF-alpha, and IL6 are produced in response to the injury and are followed by release of the neuroprotective IL-10 [34,57]. Microglia, the resident immune cells of the central nervous system, are known to have a central role in modulating this response [58]. One phase-I/II trial currently underway in China is examining the use of TNF-alpha monoclonal antibodies in acute SCI (NCT04988425). Enrollment is expected to reach 90 participants, and results are anticipated in late 2023.
Granulocyte-colony stimulating factor (G-CSF) is a cytokine glycoprotein that has increasingly been demonstrated to enhance neurogenesis and reduce TNF-alpha and IL1-beta expression levels at SCI sites [59]. Randomized phase-I/IIa studies have shown ASIA motor score improvement and a lack of significant adverse events with G-CSF therapy for acute SCI [60]. A phase-III clinical trial in Japan was recently completed with results to be published in the near future [61].
5. Nerve Regeneration Techniques
One of the most promising aims in experimental therapeutics for SCI remains establishing methods for neuronal and axonal regeneration. Current approaches focus on stem cell implantation, growth factor conditioning, neural growth inhibitor binding, tissue scaffolding, and neuromodulation. While these methods have historically been engineered to address chronic SCI, focusing regenerative efforts closer to the primary insult could theoretically expedite healing and functional recovery.
1) Stem cell implantation
The potential of stem cell therapy to induce or enhance endogenous neural regeneration and/or improve functional recovery has continued to undergo rigorous research. Stem cell therapies under investigation encompass autologous bone-marrow mononuclear cells (BM-MSCs)—including hematopoietic stem cells (HSCs) and mesenchymal stem cells—and other cell types including neural progenitor cells (NPCs), olfactory ensheathing cells (OECs), embryonic stem cells (ESCs), and induced pluripotent stem cells (iPSCs).
Bone-marrow derived mononuclear cells have the advantage of reduced immune rejection and possible synergism between HSCs and MSCs, improving angiogenesis and matrix repair [30]. On the other hand, BM-MSCs are difficult to purify and culture in vitro, and they possess limited differentiation potential [30]. Clinical trials of these therapies have shown promising but mixed results. After autologous HSC (CD-34+ and CD-133+) transplantation in 19 patients with complete SCI (ASIA-A), 37% of patients saw segmental sensory improvement (ASIA-B), 10% saw motor improvement (ASIA-C), and the remaining 53% saw no improvement (ASIA-A) within a 42–60 months follow-up period [62]. Relative to HSCs, MSCs possess higher neuronal differentiation potential, and small clinical trials have demonstrated safety and variable efficacy in improving spasticity, sensorimotor, and sphincter function in both complete and incomplete chronic SCI patients [30,63]. A meta-analysis of 328 patients demonstrated improved ASIA scores in 42% of cases after 1 year but noted the limited availability of clinical trial data and standardized methods [64]. Numerous clinical trials are ongoing, including a phase-II/III trial of MSCs in chronic ASIA-B patients by Pharmicell Co. (Seoul, Korea) and a trial of adipose-derived MSCs in ASIA-A, ASIA-B, and ASIA-C patients by Mayo Clinic. These trials were set to conclude in 2020 and 2023, respectively [65].
NPCs are native and multipotent and differentiate into neuronal-restricted precursors and glial-restricted precursors. NPC transplants readily integrate into the host milieu, induce axonal regeneration and extension, hasten remyelination, attenuate glial scar formation, and afford immunomodulation and neuroprotection [66]. A phase-I/IIa open-label nonrandomized controlled clinical trial did demonstrate AISA score improvement by at least 2 points in 5 of 19 NPC transplant patients, compared to 1 of 15 control patients [67]. Other clinical trials, such as an openlabel phase-I trial by Neuralstem (now Seneca BioPharma, Germantown, MD, USA), are ongoing. Challenges currently faced include difficult isolation of NPCs, the small number of therapy candidates, and the high cost of research [67].
OECs are glial cells that support olfactory receptor axonal growth into the olfactory bulb. Specifically, they express neurotrophins that promote neurogenesis and reduce risk of astrocyte hypertrophy. They are readily harvested autologously from the olfactory bulb and nasal mucosa and do not require patient immunosuppression. OECs are limited by inadequate source quantity and present differentiation and purification challenges similar to other regenerative options. Numerous clinical trials have been conducted, and a meta-analysis of 1,193 patients treated with OECs found no significant adverse effects, but methodological and technical concerns prevented determination of any significant efficacy as well [68]. Clinical trials in Poland (NCT03- 933072) and the United Kingdom (NCT02870426) are slated to conclude in 2023.
ESCs and iPSCs have similarly impressive differentiation potential, promising substantial efficacy; human ESCs are indeed capable of differentiation into NPCs and, subsequently, regionally specific neuronal subtypes [69]. Their pluripotency means ESCs and iPSCs do carry an associated genomic instability and risk of malignancy. The ethical stature of ESC use is also disputed. Non-human investigation of ESC- and iPSC-based treatment of SCI has demonstrated feasibility, but clinical trials are so far limited [70,71]. The Geron Corporation (Foster City, CA, USA) began a clinical trial of ESCs for SCI therapy in 2010, enrolling 5 patients before stopping the trial a year later. Not all patients enrolled had received a transplant [30]. Keio University in Tokyo, Japan, has received approval for a clinical trial of iPSC therapy for ASIA-A patients, which is ongoing [30,71].
Overall, while these data are promising in the quest to determine the best neuro-regenerative substrates in SCI, more work is required to establish efficacy and safety in the acute postinjury setting to determine long-term effects on functional recovery. Additionally, no head-to-head trials have as of yet been conducted; subsequent investigation should help to focus efforts on optimizing sourcing, delivery, and conditioning of transplanted cells.
2) Growth factor conditioning
Acidic fibroblast growth factor (aFGF) is a mitogenic, pluripotent heparin-binding protein that has been shown to enhance neuronal growth and mitigates scarring in SCI in numerous animal studies [72,73]. Human clinical trials have steadily increased in number since an initial case report demonstrating marked functional recovery of a patient with chronic SCI who was treated with 4 survival nerve grafts along with an aFGF fibrin glue and could ambulate independently with a walker at 2.5 years postinjury [74]. A different group conducted 2 more studies, 1 on 9 cervical SCI patients and a follow-up study with 60 patients (50/50 cervical and thoracolumbar SCI), and found that the use of aFGF fibrin glue resulted in significant enhancement of ASIA motor and sensory scores [75,76]. These observational studies were limited by their lack of randomization and control arms. Recombinant human aFGF (compound ES135) is currently undergoing a multicenter double blind, randomized placebo control phase-III study (NCT03229031). Hepatocyte growth factor (HGF) can enhance neuronal survival and decrease astroglial scar lesion size by increasing angiogenesis and axon regeneration [77]. Intrathecal recombinant human HGF injection has been shown to improve neurological status in rat studies [78]. Based on this, a phase-I/II trial of intrathecal recombinant HGF involving patients with acute cervical SCI found that all patients had improved Frankel scores at 72 hours [79].
3) Inhibiting neural growth inhibitors
Cethrin (VX-210) is a recombinant inhibitor of the Rho pathway which is involved in the regulation of cytoskeleton formation [30]. Inhibition of Rho has been shown in rodent thoracic contusion models to promote axonal outgrowth and improve motor function [80]. Following a phase-I/IIa clinical study that demonstrated the safety and tolerability of Cethrin for acute cervical and thoracic SCI in human patients, a double-blinded, placebo-controlled, multicenter trial (SPRING) has been developed to demonstrate therapeutic benefit, with preliminary results posted (NCT02669849).
Elezanumab is a monoclonal antibody that binds to and neutralizes repulsive guidance molecule A, an inhibitor of axonal growth and regulator of neuronal cell death [81]. A phase-II clinical trial is currently underway to evaluate the effects of Elezanumab in acute traumatic cervical SCI (NCT04295538).
4) Tissue scaffolding
Biomaterial tissue scaffolding is one promising method for facilitating SCI repair. Natural or synthetic biomaterial polymers have been shown in animal models to enhance nervous tissue regeneration by promoting survival and outgrowth of transplanted cells and providing an environment to concentrate neurotrophic growth factors [12,30]. Limited efficacy has been redemonstrated in humans. A large multicenter trial entitled INSPIRE launched in 2014 to determine the benefit of a synthetic polymer scaffold poly(lactic-co-glycolic acid)-b-poly(L-lysine) in subjects with thoracic ASIA-A impairment, has completed enrollment and expected to conclude in 2024 (NCT02138110). Multiple clinical trials led by the Chinese Academy of Sciences are investigating the application of NeuroRegen, a collagen scaffold, in concert with other experimental strategies including stem cell therapy and epidural stimulation.
Gangliosides including the glycosphingolipid ganglioside-1 (GM-1) are complex acidic glycolipids that constitute a major component of the cell membrane. Experimental evidence in animal studies has shown potential in the regeneration and growth of damaged nervous tissue leading to functional improvement [82]. One phase-II trial showed enhanced 1-year ASIA motor score improvement following daily administration of GM-1 for 18–32 days following injury [68]. However, meta-analyses evaluating potential therapeutic benefits of GM-1 across studies failed to support its widespread use for SCI [83]. Still, it has been suggested that studies with more optimized methodology (i.e., potentializing GM-1 administration with HBO and/or refining outcome measurement) may be warranted with this potential therapeutic [83].
5) Neuromodulation
Epidural stimulation (ES) is an emerging treatment strategy that has demonstrated promise in the treatment of chronic SCI in both animals and humans [84-86]. It is theorized that oscillating electrical fields may stimulate neuronal growth and remyelination and excite remaining neuronal networks at the site of the lesion [87]. Individual patients with predominantly lumbar injuries have demonstrated motor improvement with ES, including partial and full weight-bearing and overground ambulation, even with complete injuries [88,89]. Similarly promising results of upper limb motor recovery have been observed [89]. Notably, a recent study demonstrated activity-specific stimulation programs that enabled 3 individuals with complete sensorimotor paralysis to regain trunk and leg motor functions within 1 day of stimulation [90]. Several clinical trials targeting these broader functional metrics (e.g., volitional movement, autonomic function, bladder training, and sensory feedback) are underway.
FUTURE PERSPECTIVES
Table 1 summarizes the current and developing investigational therapeutic tools for treating acute SCI. Numerous methods are being developed in animal models that may soon be tested in clinical trials designed to demonstrate functional benefit in SCI. Necroptosis is a caspase-independent mechanism of programmed cell death that occurs following cellular injury in which cellular contents are not packaged into apoptotic bodies and instead leak into the extracellular space, promoting a proinflammatory state through triggering of innate and adaptive immune systems [91]. The process is dependent on receptor-interacting serine/threonine kinase 1 and 3 and studies have shown that it may be one of the primary forms of cell death following traumatic SCI [91]. Inhibition of this pathway is suspected to attenuate secondary damage and minimize cell death [92].

Summary of investigational therapeutic tools and their corresponding evidence for management of acute SCI
Autologous omental transplantation has been proposed as a method to introduce blood- and lymph-rich tissue to injured spinal cord tissue [93]. Relatively small human trials in the 1990s showed continued viability of omental grafts in chronic SCI patients but failed to demonstrate objective functional improvement, and interest in its SCI treatment potential fell flat for a time [94]. However, omental transplant therapy has progressed in other clinical settings, including flap transposition on the brain surface for neovascularization and neurotrophic effects in epileptic or ischemic brain injury [95]. Interest in omental angiogenesis as a treatment for SCI has revived in recent years, and trials in rats have improved neural preservation, reduced injury cavity, and promoted neovascularization of the injured site [93].
A newer addition to the regenerative cellular therapies discussed in the literature is peripheral nerve derived stem cells (PNSCs). Spheroid forms of PNSCs have demonstrated therapeutic potential through neurotrophic factor release and extracellular matrix expression, leading to significant functional recovery, neuronal regeneration, and neuropathic pain reduction in animal models [96].
Estrogen-based therapies are also an emerging avenue or research. Estradiol is known to provide CNS neuroprotection in animal models of SCI, traumatic brain injury, and ischemic brain injury [97]. The therapeutic capability of a third-generation selective estrogen receptor modulator, bazedoxifine, was studied in rats [98]. Bazedoxifine was shown to suppress inflammatory response and promote remyelination by inhibiting the mitogenactivated protein kinase/nuclear factor-kappa B (NF-κB) pathway and enhancing oligodendrocyte precursor cell differentiation and oligodendrocyte proliferation. There are complex and profound implications of estrogen therapy due to its natural maintenance and alteration of normal physiology. Still, there is hope that estrogen therapies may play some role in SCI treatment.
Entinostat is a class I histone deacetylase (HDAC) inhibitor directed primarily against HDAC1 and HDAC3. HDAC inhibitors have been shown to inhibit NF-κB mediated microglial activation, thus reducing SCI-induced inflammation [99]. Treatment of a mouse SCI model with Entinostat resulted in improved grip strength, Basso Mouse Scale score for locomotion, spinal edema, cell death, and local NLRP3 inflammasome activation [99].
Another method of counteracting inflammation induced by SCI may be found in IgM’s homeostatic role against IgG autoimmune response. One study found that IgM-knockout mice with induced C6–7 SCI showed significantly greater impairment in neurobehavioral recovery compared to their wild-type counterparts, including deteriorated coordination and reduced fore- and hind-limb swing speed and print-area, which serve as gait parameters [100]. IgM-knockout mice also exhibited increased lesion size, less white matter sparing, and enhanced deposition of complement-fixing IgG antibodies in the spinal cord. These newer methods will continue to be studied closely by the scientific community hoping to grow the armamentarium available to treat this multifaceted disorder.
CONCLUSION
Emerging strategies for managing SCI in the acute period stem from an evolving understanding of the pathophysiology of the disorder and focus on preventing ischemia, reducing secondary injury due to inflammation and necroptosis, modulating the cytotoxic and immune response, and promoting cellular regeneration. There is significant research ongoing in preclinical and clinical studies examining a wide array of surgical and non-surgical therapies. A major hurdle to wide acceptance of any of the emerging treatments is designing an appropriate clinical trial to test them. Continued translational research on these methods will improve the feasibility of bench-to-bedside innovations in treating patients with acute SCI.
Notes
Conflict of Interest
The authors have nothing to disclose.
Funding/Support
This study received no specific grant from any funding agency in the public, commercial, or not-for-profit sectors.
Author Contribution
Conceptualization: RG, MP, AR; Data curation: RG, DS; Funding acquisition: AR; Methodology: RG; Project administration: AR; Visualization: RG; Writing - original draft: RG, DS; Writing - review & editing: RG, DS, MP, JG, AF, AR.